Dr. Fields is Chief, Nervous System Development and Plasticity Section, National Institutes of Health, NICHD, Bethesda, Maryland. He is the author of The Other Brain: From Dementia to Schizophrenia, How New Discoveries About the Brain Are Revolutionizing Medicine and Science.
Thirty years after Albert Einstein died, sections of his brain were put under the microscope in an effort to understand his genius. Contrary to what researchers had expected, he had a typical number of neurons, but an extraordinary number of glia, a type of brain cell that had historically been viewed as having some housekeeping functions and serving as the "glue" holding the brain and its neurons together.
The discovery of Einstein's rich endowment of glia in the 1980s prompted many neuroscientists to revise their assumptions about the importance of glial cells to the brain.
Glia are nervous system cells that communicate by chemical signaling rather than by electrical impulses. Originally glia were considered connective tissue involved in neuronal support and neuroinflammation, but in the last decade it has become evident that all types of glia can sense functional activity in neurons and influence transmission of information in several ways. Glia are involved in nearly every aspect of brain function, including brain development, homeostasis, information processing, neurological disease and psychiatric illness.
Glia are far from inert interstitial brain cells. Some types of glia are involved in synaptic transmission, which implicates these glial cells in many aspects of learning, memory and other types of information processing; and in nervous system dysfunction, including neurological and psychological disorders. Other glia cells mediate immune function or form electrical insulation on nerve axons (myelin).
Glia are involved in nearly every aspect of brain function, including brain development, homeostasis, information processing, neurological disease and psychiatric illness.
Glia cannot generate action potentials, the electro-chemical signals that are the basis of neural communication. They lack the cellular structures identified with neurons, including axons, dendrites and synapses. Instead these cells exhibit a diverse range of structures consistent with their diverse functions.
It is now well established that glia use several types of chemical intercellular signals to communicate with each other and with neurons. Ions and other small molecules are spread from cell-to cell through gap junction channels coupling the cell membranes of adjacent glial cells, but glia also communicate by releasing signaling molecules. This includes many of the same neurotransmitters that neurons use for synaptic transmission, as well as growth factors, cytokines and chemokines. These chemical messages are detected by membrane receptors on other glia and on neurons. This kind of communication is more of a broadcast "wave" of neurochemical activity than the direct line connection neurons tend to use, and is shown in the video below.
- Astrocytes maintain homeostasis of neuronal function.
- Microglia fight infection and respond to injury.
- Oligodendrocytes and Schwann cells form the electrical insulation on nerve fibers (axons), which is essential for normal transmission of electrical impulses (action potentials).
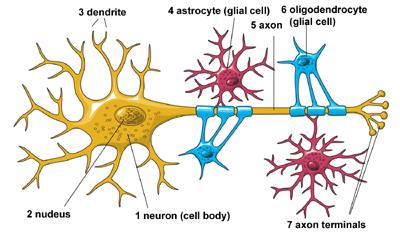
Credit: NIH/National Instititute of Neurological Disorders and Stroke (NINDS)
Astrocytes provide physical support and nutrition to neurons and respond to neural injury. The function of providing nutritional support for neurons was first deduced from the close association of some astrocytes with small blood vessels. Astrocytes near blood vessels extend processes called "end feet" that surround blood vessels, and through which substances are transported between the bloodstream and neurons.
Astrocytes have been implicated in strengthening and weakening synaptic transmission in the hippocampus in conjunction with memory formation.
Astrocytes transport ions and neurotransmitters from the extracellular space surrounding neurons to maintain the proper levels of ions and neurotransmitters. These functions are essential for maintaining the membrane potential of neurons that is necessary to fire electrical impulses and to communicate by synaptic transmission. Cellular coupling among populations of astrocytes via gap junction channels siphons away potassium ions released by electrically active axons, and disperses it through an astrocytic network for disposal into the bloodstream. Astrocytes also provide metabolic support to neurons by delivering lactate and glucose.
Astrocytes are also implicated in neural communication and appear to play a role in certain neurological diseases. Astrocytes at synapses take up neurotransmitter released by neurons. They can also release neurotransmitters and other neuroactive substances to either facilitate or inhibit synaptic communication between neurons. This enables astrocytes to respond to neuronal activity and implicates astrocytes in epilepsy and many other neurological conditions where excitability is excessive or depressed. Astrocytes have recently been shown to control excitability in the brain, affecting such behaviors as sleep and chronic pain.
Astrocytes have been implicated in strengthening and weakening synaptic transmission in the hippocampus in conjunction with memory formation. Both strengthening and weakening of synapses can be regulated by astrocytes in the hippocampus through the release of neurotransmitters, notably glutamate, ATP and D-serine, but also by the delivery of glucose to neurons and by the maintenance of normal concentrations of extracellular ions (notably potassium ions), and glutamate.
In addition to releasing neurotransmitters, astrocytes release many types of growth factors, cytokines and antioxidants that protect and stimulate the growth of neurons. Unfortunately,under pathological or disease conditions, astrocytes can release oxidizing compounds, neurotransmitters, inflammatory cytokines and other toxic substances that damage or kill neurons. These actions involve astrocytes in neurological disorders such as ALS, Parkinson's disease and Alzheimer's disease.
Oligodendrocytes are the major reason why damaged axons in the spinal cord and brain cannot regenerate.
The myelin sheath on axons changes the way impulses are transmitted, accelerating transmission speed approximately 50 times faster than in unmyelinated axons of the same diameter. Rather than propagating continuously down the axon, as in axons that lack myelin, the impulse (action potential) is generated at bare regions between adjacent segments of axons that are myelinated. These are the nodes of Ranvier, where sodium channels are highly concentrated, and where an action potential is generated upon depolarization to induce an impulse in sequential nodes, much like repeater stations in a communication system. Only vertebrates have myelin.
Proteins in the myelin sheath formed by oligodendrocytes, such as Nogo-A and others, strongly inhibit the growth and sprouting of damaged axons in the central nervous system (CNS). These inhibitory proteins stabilize neural circuits in the brain after they have formed and been remodeled by functional activity that is driven by environmental experience and learning. Thus, myelination contributes to closing the critical period for learning. Unfortunately, however, these myelin proteins inhibit regeneration of axons after injury; thus oligodendrocytes are the major reason why damaged axons in the spinal cord and brain cannot regenerate.
Microscopic studies, analyses of gene expression and brain imaging have demonstrated that oligodendrocytes are linked to mental illness. Several genes in oligodendrocytes or that regulate oligodencrocyte development and myelination are expressed at abnormally low levels in brain tissue from people with schizophrenia and chronic depression, and a number of these oligodendrocyte gene variants have been identified as risk factors for these mental illnesses.
MRI brain imaging of people learning complex skills such as learning to read, play the piano or juggle show that there are changes in glia-rich white matter regions of the brain . White matter tracts in the brain are regions where myelinated axons form bundles of fibers connecting neurons in gray matter regions into circuits. Increasing the number of myelinated axons or modifying axons that are already myelinated could improve performance by optimizing the transmission of impulses between cortical regions mediating complex cognitive functions.
The same effects on transmission speed and synchrony could involve white matter in cognitive dysfunctions, such as dyslexia, ADHD and psychiatric illnesses, that are associated with disorganized or abnormal processing of cognitive function controlling thoughts, moods and control of behavior.
Recent research has shown that electrical impulses in axons can be detected by oligodendrocytes, and several cellular and molecular mechanisms for the activity-dependent communication between axons and oligodendrocytes have been identified thus far. These studies in cell culture have shown that action potentials can regulate glial cell proliferation, development and stimulate myelination of unmyelinated axons.
Under normal physiological conditions microglia remove synapses in response to neuronal activity to modify neural circuits appropriately to environmental experience, and microglia have been implicated in chronic pain and psychiatric disorders, such as OCD, by releasing neuromodulatory substances, including cytokines, neurotransmitters, nitric oxide, ATP and others (for review see ).
Astrocytes and microglia are the "first responders" to brain injury; they participate in scar formation, immune defense, and clearing and remodeling damaged tissue. The defensive actions of astrocytes and microglia also implicate them in the cognitive decline seen in aging. Astrocytes can contribute to generating the toxic amyloid plaques that form in Alzheimer's disease, and microglia remove the toxic plaques. Both types of glial cells can be impaired in their normal functions when they become damaged in Alzheimer's disease. A significant proportion of normal tissue loss in the aging brain results from the loss of white matter formed by oligodendrocytes.
The defensive actions of astrocytes and microglia also implicate them in the cognitive decline seen in aging.
In contrast to neurons, which cannot undergo cell division after maturation, many types of glia can divide and differentiate into other kinds of brain cells. . Some glial progenitors can divide and turn into oligodendrocytes, astrocytes, neurons or undifferentiated cells with the potential to generate various types of brain cells. With this capability, glia respond to nervous system injury and disease, and can also replace brain cells lost with age. However, this "stem-cell-like" property of glia also implicates them in brain cancer, as nearly all cancers originating in the brain derive from types of glial cells.
Many infectious diseases attack glial cells and the loss of normal glial function results in neuronal degeneration or dysfunction. HIV, for example, can cause dementia, with the virus infecting microglia and astrocytes but not neurons.
Other neurological diseases result from direct effects on glia. Multiple sclerosis is an autoimmune disorder that attacks the oligodendrocytes that form the myelin insulation on nerve fibers. The resultant myelin damage severely interrupts normal impulse transmission leading to significant deficits in sensory, motor and some cognitive function. Axons that lose their myelin sheath can die, demonstrating the high degree of dependence of neurons on glial function.
Several cellular functions performed by glia involve them in many cognitive functions including dysfunctional human behavior. As indicated briefly above, all types of glia have been implicated in various types of psychiatric illness. The role of astrocytes in regulating neurotransmitter levels at synapses is an example of how glia participate in mental illness and suggests the need for further research.
How electroconvulsive shock treatment works is still unclear, but the release of growth factors, neurotransmitters and stimulation of neurogenesis may be involved, and astrocytes participate in all of these processes.
Most pharmacological treatments for mental illness are based on regulating neurotransmission. SSRIs (selective serotonin reuptake inhibitors) used in the treatment of chronic depression, for example, regulate the levels of the neurotransmitter serotonin in the synaptic cleft by inhibiting the re-uptake of the neurotransmitter once it is released. Astrocytes at synapses are the cells that normally perform this neurotransmitter clearing function together with neurons. Many psychoactive drugs act by modulating neurotransmitter function, and psychotic behaviors of individuals under the influence of these compounds cannot be easily distinguished from many psychotic behaviors exhibited by people with certain mental illnesses such as schizophrenia. In theory, similar cognitive effects would occur if astrocytes fail to properly regulate neurotransmitter levels.
Changes in astrocytes are seen in postmortem tissue of people with various mental illnesses. The decrease in number of astrocytes in the cerebral cortex of people with chronic depression and schizophrenia, observed by Ladislav von Meduna in the 1930s, was the inspiration that led to electroconvulsive shock treatment, still the most effective treatment for chronic depression that cannot be relieved by medications.
In epilepsy, the number of astrocytes is increased and the astrocytes develop a more robust morphology. Therapeutically increasing the number of astrocytes in the cerebral cortex of people suffering chronic depression or schizophrenia by inducing seizure was proposed by Meduna in 1935 to correct this cellular imbalance. How electroconvulsive shock treatment works is still unclear, but the release of growth factors, neurotransmitters and stimulation of neurogenesis may be involved, and astrocytes participate in all of these processes.
The glial brain and the neuronal brain work differently but in a close association that is essential for brain function. Glia communicate slowly relative to rapid signaling between neurons. They communicate by broadcasting chemical signals widely rather than signaling serially via discrete points of contact as neurons do as they communicate through chains of synapses.
This means that a single astrocyte can cover large areas of the brain encompassing thousands of synapses. These broadcast features implicate glia in slowly changing nervous system processes having a more general influence on the brain. For example, astrocytes regulate the hormones secreted to regulate thirst, lactation and the maintenance of general levels of excitability in the brain.
We also now know that white matter changes with learning. Research on “learning” and memory will now necessarily need to go beyond the mechanisms of neurotransmitter function at synapses and begin to consider the subtler, more pervasive actions of glia.
Glia perform far more functions in the brain than neurons. Neurons are elegant cells, highly specialized for rapid transmission and integration of information, but most of the brain's functions are carried out by cells that have been comparatively neglected by researchers until recently—glia.
Fields, R. D. (2004) The other half of the brain. Scientific American April 290, 54-61.
Fields, R. D. (2008) White matter in learning, cognition, and psychiatric disorders, Trends in Neuroscience 31, 361-370.
Fields, R. D. (2008) White matter matters. Scientific American March, 298, 54-56.
Fields, R. D. (2009) New culprits in chronic pain. Scientific American, November 301, 50-57.
Fields, R. D. (2010) The Other Brain, New York, Simon and Schuster.
Fields, R.D. and Stevens-Graham, B. (2002) New insights into neuron-glia communication. Science 298, 556-562.
Fields, R.D. (2011) The Hidden Brain, Scientific American Mind, May/June, p. 53-59
Kettenmann, H. T. and Ransom, B. R. (2005) Neuroglia (2nd Edition). Oxford, Oxford University Press.
Wake, H., Lee, P.R., and Fields, R.D. (2011) Control of local protein synthesis and initial events in myelination by action potentials. Science 333: 1647-51.
Schafer, D.P., Lehrman, E.K., Kautzman, A.G., Koyama, R., Mardinly, A.R., Yamasaki, R., Ransohoff, R.M., Greenberg, M.E., Barres, B.A., Stevens, B. (2012) Microglia sculpt postnatal neural circuits in an activity and complement-dependent manner. Neuron 74: 691-7705.